Key Factors Influencing Heat Transfer and Combustion Efficiency in Industrial Power Plant Boilers
Key Factors Influencing Heat Transfer and Combustion Efficiency in Industrial Power Plant Boilers
In power generation, industrial power plant boilers are at the core of converting fuel energy into electrical or mechanical power. Achieving high combustion and heat transfer efficiency is essential not only for maximizing output but also for minimizing fuel costs, emissions, and equipment wear. Inefficient combustion or poor heat transfer can result in fuel wastage, reduced output, boiler fouling, and unplanned shutdowns. Understanding the key influencing factors allows operators to optimize performance, reduce operating costs, and meet environmental targets.
The heat transfer and combustion efficiency in industrial power plant boilers are influenced by factors such as fuel quality, air-to-fuel ratio, furnace and burner design, flue gas temperature, slagging and fouling behavior, ash content, and the condition of heat exchanger surfaces. Effective combustion requires precise control of air supply and fuel delivery, while high heat transfer efficiency depends on clean and well-maintained heating surfaces, appropriate boiler loading, and balanced flue gas velocities.
Let’s break down the critical parameters that determine how efficiently power plant boilers operate.
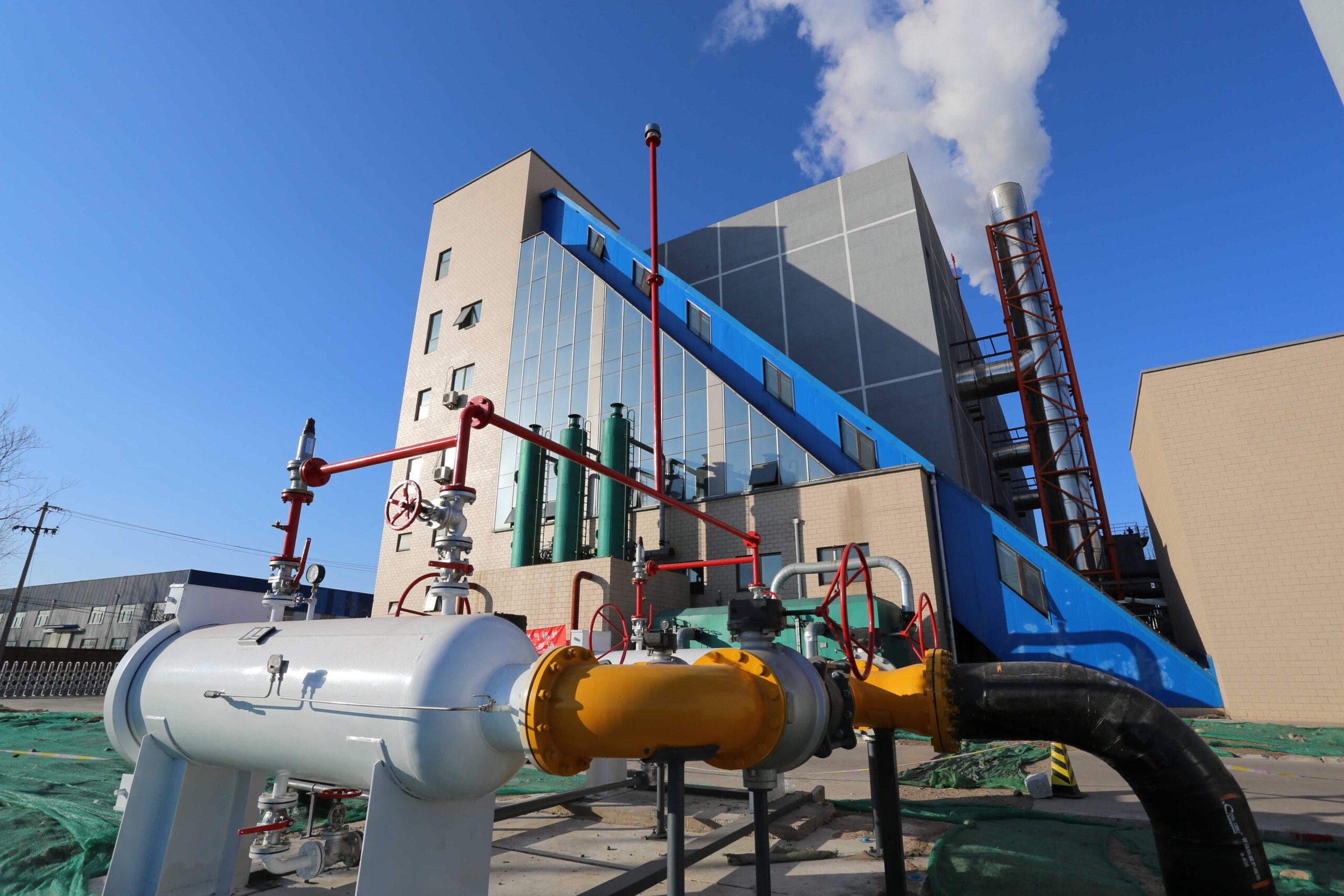
How Does Fuel Type and Calorific Value Affect Combustion Efficiency in Power Boilers?
In power boilers, not all fuels are created equal. Different fuels bring different combustion characteristics, chemical compositions, and energy contents—making them perform very differently under identical conditions. Whether it’s natural gas, coal, biomass, or waste-derived fuels, the type of fuel and its calorific value (CV) directly impact flame temperature, combustion duration, fuel handling requirements, and ultimately, boiler efficiency. Poorly matched fuels can cause incomplete combustion, high emissions, and frequent maintenance, while properly matched fuels support cleaner, more efficient energy conversion.
Fuel type and calorific value affect combustion efficiency by determining how much energy is released per unit of fuel, how completely it can be burned, and how it interacts with boiler heat transfer surfaces. High-calorific fuels provide more thermal energy per unit, reducing fuel input and increasing flame stability. Low-calorific fuels often require higher mass flow, produce more ash, and lower flame temperature, which reduces combustion completeness and efficiency. Matching boiler design and burner configuration to the fuel’s properties ensures optimal heat release and minimal losses.
To maximize efficiency, it’s not just about burning fuel—it’s about burning the right fuel in the right way.
The calorific value of a fuel directly influences how efficiently it can be converted to heat in a boiler.True
Higher calorific value fuels produce more energy per unit mass, enabling higher flame temperatures and more complete combustion.
🔥 What Is Calorific Value (CV) and Why It Matters
Calorific value is the amount of heat energy released when a given quantity of fuel is burned completely. It is typically expressed in MJ/kg or BTU/lb.
Fuel Type | Calorific Value (MJ/kg) | Combustion Implication |
---|---|---|
Natural Gas | 48–50 | High efficiency, clean combustion |
Bituminous Coal | 24–30 | Good flame stability, high ash |
Biomass (dry wood) | 16–19 | Moderate efficiency, renewable |
Wet Biomass | 8–14 | Low flame temp, requires drying |
RDF (Refuse-Derived Fuel) | 10–18 | Variable, often inconsistent composition |
The higher the CV, the less mass is needed to deliver a specific amount of energy—resulting in better heat transfer, lower flue gas volume, and reduced emissions.
Low-calorific fuels like wet biomass increase boiler efficiency due to slower combustion.False
Low-CV fuels often reduce combustion temperature and increase the fuel mass needed, lowering efficiency and increasing handling requirements.
📉 How Fuel Type Affects Combustion Efficiency
Fuel Property | Effect on Combustion | Efficiency Impact |
---|---|---|
Moisture Content | Absorbs heat to evaporate water | Lowers flame temp and efficiency |
Volatile Matter | Affects ignition and burn rate | High volatiles = faster, more complete burn |
Ash Content | Increases inert load, reduces heat transfer | Lowers effective heat utilization |
Sulfur/Nitrogen | Affects emissions and burner tuning | Requires additional air or sorbents |
Particle Size | Determines surface area for reaction | Too coarse = unburned fuel losses |
Fuels with high moisture or ash significantly reduce combustion zone temperature and heat exchange efficiency—making them more expensive per unit of usable energy.
📊 Comparison of Fuel Performance in Power Boilers
Fuel Type | CV (MJ/kg) | Ash (%) | Moisture (%) | Typical Efficiency (%) |
---|---|---|---|---|
Natural Gas | ~50 | 0 | 0 | 92–94% |
Bituminous Coal | 25–30 | 10–15 | 5–10 | 83–88% |
Dry Biomass | 17–19 | 1–5 | 10–15 | 78–85% |
Wet Biomass | 10–14 | 1–5 | 30–50 | 65–75% |
RDF | 12–18 | 15–25 | 10–20 | 70–80% |
Lower-CV fuels require higher fuel feed rates, increase flue gas volume, and often lead to flame instability, especially during load swings.
Combustion efficiency is not affected by fuel composition as long as temperature is high enough.False
Fuel composition affects flame shape, ignition, burn time, and ash formation—all of which influence combustion efficiency.
🔧 Matching Fuel to Boiler Design
Boiler Type | Best-Suited Fuel Types | Why It Works Well |
---|---|---|
Pulverized Coal Boiler | Bituminous/sub-bituminous coal | High burn rate, stable flame |
CFB Boiler | Biomass, coal, sludge, RDF blends | Handles high ash/moisture, long residence time |
Grate-Fired Boiler | Wood chips, bark, agricultural waste | Good for coarse and variable fuels |
Gas-Fired Water Tube | Natural gas, propane | Clean, fast-response combustion |
Boilers must be optimized for the physical and chemical nature of the fuel to ensure full combustion and high heat transfer rates.
🧪 Case Study: Biomass vs. Coal Comparison in 50 MW Boiler
Scenario 1 – Coal-Fired Operation:
Fuel: Bituminous coal, CV: 27 MJ/kg
Efficiency: 87.5%
Ash content: 11%
Stack temperature: 170°C
Scenario 2 – Biomass-Fired Operation:
Fuel: Wood chips, CV: 16.5 MJ/kg
Efficiency: 79.3%
Ash content: 2%
Stack temperature: 210°C
Findings:
Required 48% more biomass by mass to match load
Increased flue gas volume by 33%
Reduced efficiency due to lower flame temp and higher stack loss
Switching from coal to biomass without adjusting combustion settings can lead to efficiency losses.True
Biomass has different burn characteristics and requires tuning of air/fuel ratio, burner settings, and heat exchange strategies.
📋 Combustion Optimization Tips by Fuel Type
Fuel Type | Optimization Strategy | Purpose |
---|---|---|
Coal | Pulverize for uniform ignition, manage slagging | Improve surface area and combustion control |
Biomass | Pre-dry or blend with drier fuel | Boost CV and flame stability |
RDF | Screen and homogenize composition | Reduce volatile peaks and ash fouling |
Natural Gas | Use modulating burners and O₂ trim | Ensure tight control and low excess air |
Fuel properties also affect burner design, combustion air distribution, and emission controls—all of which are critical to maintaining high efficiency.
Summary
The type of fuel and its calorific value are fundamental factors in the combustion efficiency of power boilers. High-CV, low-ash fuels like natural gas produce more heat per unit and support stable, clean combustion. Lower-CV fuels like wet biomass require more mass, reduce flame temperatures, and increase stack losses, lowering system efficiency. By selecting appropriate fuels, pre-treating when necessary, and aligning combustion strategies with fuel characteristics, operators can maximize energy output, minimize emissions, and control operating costs. In power generation, choosing the right fuel is not just about supply—it’s about efficiency and sustainability.
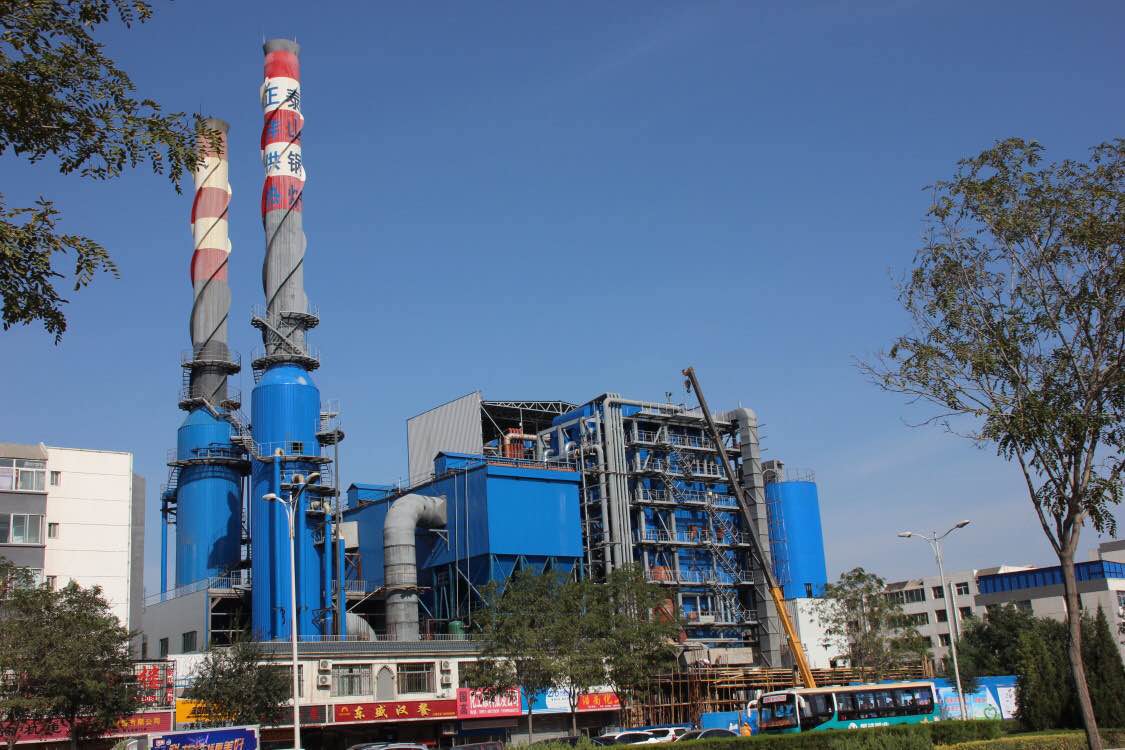
Why Is Controlling Excess Air and Air-Fuel Ratio Crucial for Complete Combustion?
In industrial combustion systems, achieving complete combustion means converting all fuel into usable heat while minimizing emissions. But combustion doesn’t happen automatically—it depends on having the right balance of fuel and air. Too little air leads to unburned fuel and hazardous emissions. Too much air cools the flame and wastes heat energy through the flue gas. Therefore, precise control of the air-fuel ratio and excess air is not optional—it’s essential for efficient, safe, and clean combustion.
Controlling excess air and air-fuel ratio is crucial for complete combustion because it ensures that every unit of fuel is fully oxidized, maximizing energy output while minimizing emissions. The correct air-fuel ratio supplies just enough oxygen to burn all fuel molecules. A slight amount of excess air (typically 10–20%) is used to compensate for mixing inefficiencies. Too little air causes incomplete combustion, resulting in carbon monoxide and unburned hydrocarbons. Too much air lowers flame temperature and increases stack losses. Maintaining the optimal ratio ensures stable flame, high thermal efficiency, and reduced environmental impact.
In combustion, precision equals performance—and the air-fuel balance is your most powerful tool.
Controlling excess air is necessary to achieve complete combustion and maximize thermal efficiency.True
Correct excess air ensures all fuel is burned while minimizing heat loss and emissions, which are both impacted by poor air control.
🔬 Stoichiometric Combustion: The Ideal Balance
At the stoichiometric point, the air supply contains exactly enough oxygen to react with all the fuel without leaving unburned fuel or unused oxygen.
Fuel Type | Stoichiometric Air-Fuel Ratio (mass) | O₂ in Flue Gas (Ideal) |
---|---|---|
Natural Gas | 17.2:1 | ~2% |
Fuel Oil | 14.5:1 | ~3% |
Coal | 10.5–11.5:1 | ~4% |
Biomass | 5–9:1 (varies with moisture) | ~5–8% |
However, in real-world applications, a slight amount of excess air is necessary to compensate for imperfect mixing and ensure complete combustion.
Operating exactly at the stoichiometric point ensures maximum combustion efficiency.False
Real systems require some excess air to avoid incomplete combustion caused by mixing limitations and air-fuel imbalances.
📉 Effects of Air-Fuel Imbalance
Condition | Flame Behavior | Efficiency Impact | Emissions Risk |
---|---|---|---|
Fuel-Rich (Low Air) | Yellow, smoky flame | Incomplete combustion, energy loss | High CO, soot, unburned hydrocarbons |
Stoichiometric | Hot, stable blue flame | Maximum thermal energy | Minimal emissions |
Excess Air (Lean) | Cool, slow flame | Heat loss through flue gas | Higher NOₓ, unburned O₂ |
Excess air reduces flame temperature and increases the mass of flue gas, which leads to higher stack temperatures and reduced heat transfer efficiency.
📊 Efficiency Loss from Excess Air
Excess Air (%) | Flue Gas O₂ (%) | Approx. Efficiency Loss (%) |
---|---|---|
10 | ~2 | 0.5 |
25 | ~4 | 1.5–2 |
50 | ~8 | 3–5 |
100 | ~11 | 5–8 |
Each additional 1% of O₂ in the flue gas above ideal corresponds to approximately 0.5–1% loss in thermal efficiency, especially in high-temperature applications like power boilers.
High excess air levels increase boiler thermal efficiency by ensuring complete combustion.False
Too much excess air cools the flame and carries heat out through the stack, decreasing overall efficiency.
🔧 Tools and Techniques for Controlling Air-Fuel Ratio
Technology | Role in Optimization |
---|---|
Oxygen Trim Controllers | Adjust airflow in real time based on flue gas O₂ |
CO/CO₂ Monitoring | Detect incomplete combustion |
Mass Flowmeters | Ensure accurate fuel and air delivery |
PID Control Systems | Maintain stable air-fuel balance |
Burner Tuning | Calibrate for specific fuels and conditions |
Automated combustion control systems combine these tools to dynamically maintain the optimal ratio, even as load or fuel quality changes.
🧪 Case Study: Industrial Steam Boiler Optimization
A 15 TPH gas-fired boiler at a food processing plant exhibited:
Flue gas O₂ at 6.5%
Stack temperature: 245°C
High fuel use at low loads
Actions:
Installed oxygen trim control
Tuned burner air/fuel curve
Adjusted damper automation
Results:
O₂ reduced to 3.2%
Stack temperature dropped to 195°C
Combustion efficiency increased by 7.4%
Annual fuel savings: ~$58,000
CO emissions reduced by 60%
Oxygen trim systems adjust combustion air based on real-time flue gas feedback.True
This closed-loop system maintains optimal air-fuel ratios dynamically, preventing combustion drift.
📋 Real-World Benefits of Air-Fuel Ratio Control
Benefit | Explanation |
---|---|
Lower Fuel Consumption | Less excess air = more energy retained |
Improved Flame Stability | Stable combustion reduces cycling and flameout |
Reduced Emissions | Less CO, NOₓ, and unburned hydrocarbons |
Higher Heat Transfer | Higher flame temperature = better efficiency |
Increased Equipment Life | Fewer hot/cold cycles and corrosion risks |
These benefits compound over time, especially in large-scale or continuous operation systems.
Summary
Controlling excess air and the air-fuel ratio is one of the most effective ways to achieve complete combustion and optimize boiler performance. It ensures that all fuel is efficiently converted to heat while minimizing waste and emissions. By preventing both under- and over-aeration, operators can achieve the sweet spot of high combustion efficiency, low flue gas loss, and consistent operation. In any combustion system, air-fuel balance is the heartbeat of efficiency—and controlling it is key to clean, cost-effective energy conversion.
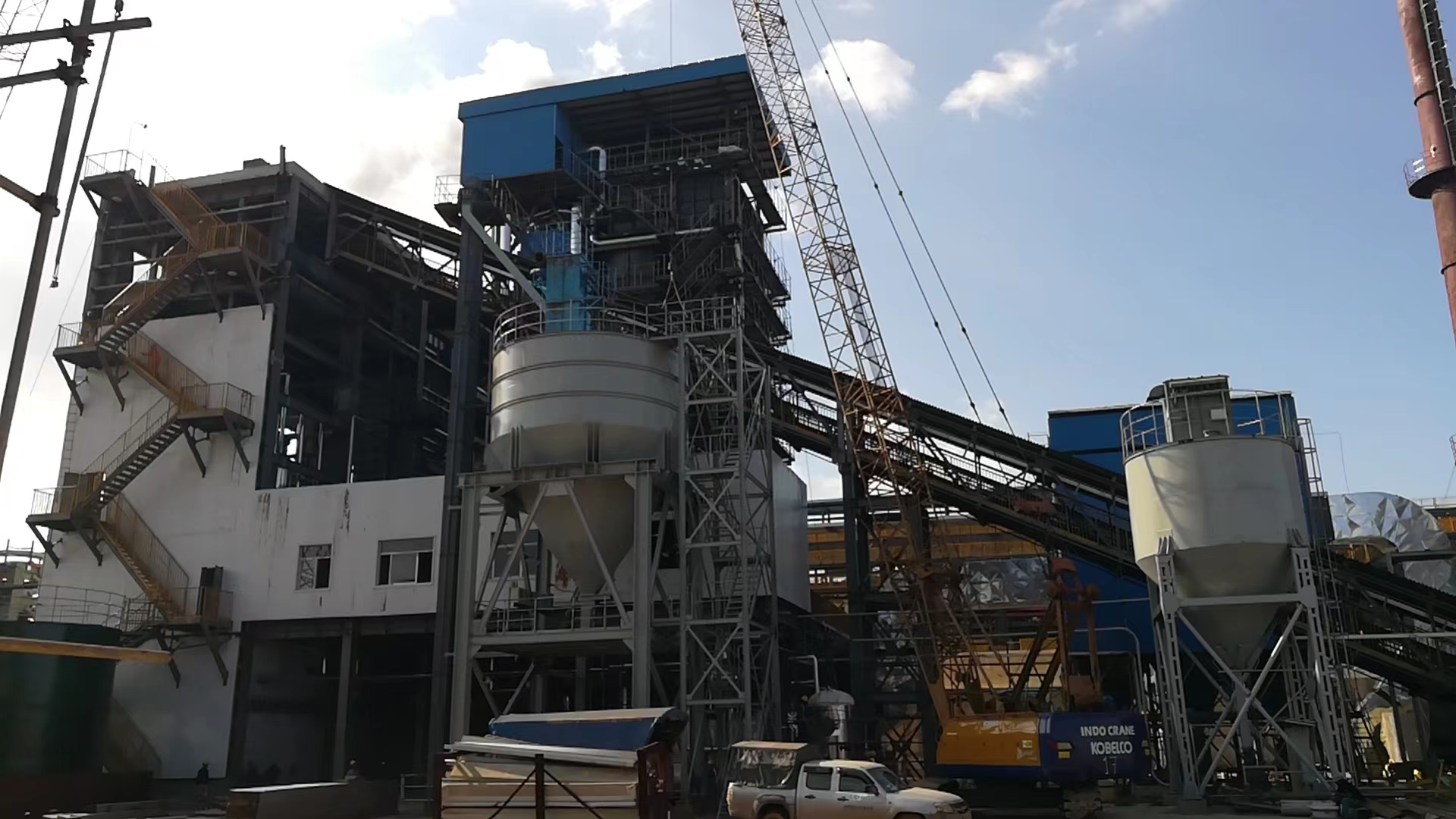
How Do Slagging, Fouling, and Ash Content Impact Heat Transfer Surfaces and Efficiency?
In high-temperature combustion systems, especially those burning solid fuels like coal, biomass, or waste, ash is not just a byproduct—it’s a performance-limiting factor. Ash particles from fuel impurities can deposit on heat transfer surfaces, forming insulating layers that degrade efficiency. In extreme cases, ash melts and forms molten slag, leading to tube erosion, overheating, and system failures. Slagging, fouling, and ash content are therefore critical variables that directly influence boiler performance, fuel costs, and operational reliability.
Slagging, fouling, and ash content impact heat transfer efficiency by forming insulating deposits on boiler surfaces, reducing thermal conductivity and impeding heat flow. Slagging refers to molten or partially molten ash that solidifies on high-temperature surfaces, while fouling is the accumulation of dry, sticky particulates in lower-temperature zones. These deposits reduce the effective surface area for heat exchange, increase flue gas temperatures, elevate pressure drops, and force boilers to work harder to meet demand. High ash content in fuel increases the frequency and severity of these issues, lowering combustion efficiency and raising maintenance costs.
In thermal systems, a clean surface is a performing surface—and managing ash behavior is key to sustaining efficiency.
Ash deposits on boiler surfaces reduce heat transfer efficiency and increase fuel consumption.True
Ash acts as an insulating layer, forcing more fuel to be burned to achieve the same heat output.
🧱 What Are Slagging and Fouling?
Term | Zone Affected | Description |
---|---|---|
Slagging | Furnace and superheater | Molten ash fuses to surfaces and hardens |
Fouling | Economizer, convection zone | Dry ash particles stick to cooler surfaces |
Ash Content | Fuel property | Inorganic residue that does not combust, becomes slag/fouling |
Slagging occurs at temperatures above 1,200°C, often around burner nozzles and flame cores. Fouling happens downstream, typically between 350–650°C, in areas like economizers and air preheaters.
📉 Efficiency Loss from Deposits
Deposit Thickness (mm) | Heat Transfer Loss (%) | Flue Gas Temp Increase (°C) | Efficiency Loss (%) |
---|---|---|---|
0.5 | 4–6% | +25–40 | 2–3% |
1.0 | 10–12% | +50–70 | 4–6% |
2.0 | >20% | +80–120 | 7–10% |
Even a thin ash layer of 1 mm can reduce boiler heat transfer efficiency by more than 10%.True
Ash insulates the tube surface, hindering heat flow into the fluid and increasing stack losses.
🧪 Case Study: Biomass CFB Slagging Impact
A 45 MW CFB boiler burning agricultural waste had:
Ash content: ~16%
High alkali content (K, Na) → low ash fusion point
Superheater slagging every 3–4 weeks
Impact:
Superheater heat transfer dropped by 18%
Flue gas temperature rose from 210°C to 290°C
Steam output dropped by 7.5%
Efficiency loss: ~6.2%
Cleaning downtime: ~8 hours/month
After switching to a lower-alkali fuel blend and installing soot blowers:
Cleaning frequency reduced by 60%
Efficiency improved by 5.4%
Annual savings: $73,000 in fuel and labor
🔬 Key Fuel Properties Affecting Slagging and Fouling
Property | Influence | Impact on Performance |
---|---|---|
Ash Content (%) | More residue post-combustion | More frequent cleaning, wear, heat loss |
Ash Fusion Temperature | Lower values → slagging risk | Causes melting, clinkering, blocked tubes |
Alkali Metals (K, Na) | React with silica to form slag | Promotes sticky deposits and tube corrosion |
Volatile Matter | Affects flame shape and ash location | Alters where fouling occurs |
Chlorine Content | Corrosive ash and slag formation | Shortens equipment lifespan |
Fuels with high alkali and chlorine levels are notorious for fouling and slagging in biomass and RDF boilers.
High alkali content in biomass increases slagging risk in high-temperature combustion zones.True
Alkali metals react with silicates to form low-melting-point slag, which adheres to hot surfaces.
🔧 Mitigation Strategies for Ash-Related Issues
Strategy | Purpose | Implementation Frequency |
---|---|---|
Fuel Blending | Reduces ash content and balances alkalis | Based on fuel availability |
Soot Blowers | Dislodge fouling layers | Daily or per shift |
Ash Fusion Testing | Predicts slagging risk | Quarterly or per batch |
Additive Injection | Binds ash or changes melting behavior | Continuous |
Automated Cleaning Systems | Remote tube cleaning during operation | Weekly or as needed |
Boiler operators often implement a combination of strategies to balance performance, cost, and downtime.
📊 Ash Content vs. Cleaning Frequency
Fuel Type | Ash Content (%) | Typical Cleaning Frequency |
---|---|---|
Natural Gas | 0% | Rare/None |
Pulverized Coal | 10–20% | Weekly to biweekly |
Dry Biomass | 2–6% | Weekly |
Agricultural Waste | 15–30% | Daily or continuous |
RDF | 20–25% | Daily + monthly deep cleaning |
Fuels with higher ash content not only reduce combustion efficiency but significantly increase O&M workload and cost.
Switching to low-ash fuels can reduce boiler maintenance costs and improve heat transfer efficiency.True
Less ash means fewer deposits, better heat absorption, and lower cleaning frequency.
🧠 Smart Monitoring and Predictive Cleaning
Modern plants use diagnostic tools to manage slagging and fouling proactively.
Tool/Technology | Use Case |
---|---|
Infrared Cameras | Detect surface hot spots and insulation loss |
Tube Wall Thermocouples | Track deposit growth by temperature gradient |
Flue Gas Temperature Sensors | Monitor stack loss trends |
AI Pattern Recognition | Predict fouling zones before heat drop |
Acoustic Cleaning Systems | Dislodge ash with sound waves |
These tools allow operators to clean only when necessary, preserving boiler uptime and avoiding energy loss.
Summary
Slagging, fouling, and ash content are major factors affecting heat transfer and combustion efficiency in power boilers. Deposits act as insulators that hinder thermal flow, raise flue gas temperatures, and force the boiler to work harder to maintain output. High ash fuels increase cleaning frequency, maintenance burden, and fuel costs. By selecting appropriate fuels, monitoring ash behavior, and implementing proactive cleaning strategies, facilities can maintain clean heat surfaces, minimize energy loss, and reduce downtime. In combustion systems, efficiency is only as good as the cleanliness of the surfaces that transfer heat.
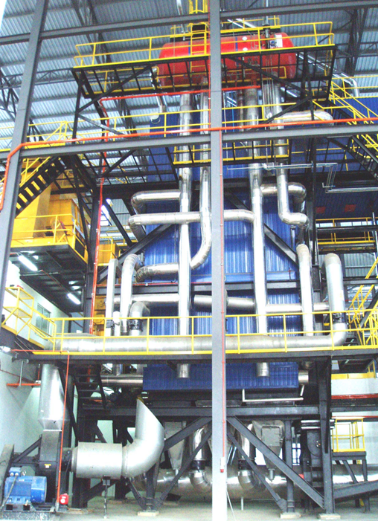
What Is the Role of Flue Gas Temperature in Identifying Heat Losses and Boiler Performance?
In every fuel-burning boiler, not all the energy released from combustion is captured. A significant portion escapes with the hot flue gases, making flue gas temperature a key diagnostic indicator of boiler efficiency and energy loss. By analyzing this temperature at the stack, operators can evaluate how effectively heat is transferred from combustion gases to the working fluid (steam or hot water). Higher-than-expected flue gas temperatures often signal poor heat absorption due to fouling, excess air, poor tuning, or under-loading. Conversely, lower (but safe) flue gas temperatures generally indicate optimal heat recovery.
Flue gas temperature plays a critical role in identifying heat losses and assessing boiler performance by revealing how much thermal energy is leaving the system unutilized. When flue gases exit the stack at high temperatures, it indicates that not enough heat has been transferred to the fluid in the boiler. This represents a direct loss of combustion energy, reducing overall thermal efficiency. Monitoring flue gas temperature helps detect inefficiencies caused by fouling, excess air, low boiler load, or poor burner performance—allowing timely corrective actions that restore efficiency and reduce fuel costs.
Simply put, the hotter your flue gas, the colder your profits.
High flue gas temperature indicates energy is being lost through the stack and suggests poor heat recovery.True
When flue gases retain too much heat, it means less thermal energy has been transferred to the process fluid, reducing efficiency.
🌡️ What Is a Normal Flue Gas Temperature?
Boiler Type | Optimal Flue Gas Temp (°C) | Caution Level (°C) | Action Threshold (°C) |
---|---|---|---|
Natural Gas Boiler | 120–160 | >180 | >200 |
Oil-Fired Boiler | 170–220 | >240 | >260 |
Coal-Fired Boiler | 180–250 | >270 | >300 |
Biomass Boiler | 150–230 | >250 | >280 |
Ideal flue gas temperatures depend on the fuel type, boiler design, and heat recovery systems. Condensing boilers, for example, aim for stack temperatures below the dew point (~55–65°C for natural gas) to reclaim latent heat.
📉 Efficiency Impact of Flue Gas Temperature
Flue Gas Temp (°C) | Approx. Efficiency (%) | Heat Loss via Stack (%) |
---|---|---|
120 | ~91–93% | 5–6% |
160 | ~88–90% | 7–9% |
200 | ~85–87% | 10–12% |
250 | ~80–83% | 14–17% |
300 | <78% | >18% |
Every 10°C increase in flue gas temperature corresponds to a 0.5–1% drop in boiler efficiency, especially in mid- to high-efficiency systems.
Reducing flue gas temperature too much can cause corrosion due to acid dew point condensation.True
If flue gas cools below the dew point (especially in sulfur-rich fuels), acidic condensates can form and damage boiler surfaces.
🔧 Causes of Elevated Flue Gas Temperatures
Cause | Description | Efficiency Impact |
---|---|---|
Fouled Heat Surfaces | Soot, scale, or ash insulation | Blocks heat flow to water/steam |
Excess Combustion Air | More air = more mass to heat | Cools flame, increases stack heat loss |
Poor Burner Tuning | Flame not directed at transfer surfaces | Reduced effective heat transfer |
Under-Loaded Boiler | Less heat absorbed than designed | High stack losses due to short residence time |
Bypassed Economizer | Less heat recovery | Higher final gas temperature |
📊 Flue Gas Temp vs. Stack Loss Chart
Flue Gas Temp (°C) | Excess Air (%) | Fuel Type | Stack Heat Loss (%) |
---|---|---|---|
160 | 10 | Natural Gas | 7.6 |
200 | 15 | Fuel Oil | 11.2 |
250 | 25 | Bituminous Coal | 15.9 |
300 | 30 | Biomass | 20.4 |
Stack heat loss is a function of temperature and excess air, both of which should be minimized within safe limits.
Excess air and high flue gas temperature are directly correlated with stack heat losses.True
More air and hotter exhaust mean more energy leaves the boiler without doing useful work.
🧪 Case Study: Food Plant Boiler Optimization
A 10 TPH steam boiler at a dairy facility burned natural gas and showed:
Flue gas temperature: 245°C
O₂ levels: 6.8%
Fuel usage: 12,800 m³/day
Actions Taken:
Installed oxygen trim control
Added economizer to recover flue heat
Cleaned soot from fire-side tubes
Results:
Flue gas temp reduced to 165°C
O₂ trimmed to 3.5%
Boiler efficiency improved by 7.3%
Annual fuel savings: ~$49,500
📋 Tools for Monitoring Flue Gas Temperature
Tool/Instrument | Purpose |
---|---|
Stack Thermocouples | Continuous flue gas temp measurement |
Infrared Thermography | Detects surface heat anomalies on boilers |
Flue Gas Analyzers | Monitor O₂, CO, and temperature |
Smart Economizer Control | Modulates flow based on real-time temperature |
SCADA/PLC Integration | Tracks trends and triggers efficiency alerts |
Combining flue gas temperature with O₂ and CO data provides a complete picture of combustion quality and heat recovery effectiveness.
Summary
Flue gas temperature is a powerful and immediate indicator of how well a boiler is capturing and using the energy from combustion. High flue gas temperatures signal lost heat, reduced efficiency, and potential maintenance issues, while lower, stable temperatures indicate good heat transfer and optimized operation. By monitoring and minimizing flue gas temperature through tuning, cleaning, and heat recovery systems, operators can significantly reduce fuel consumption, lower emissions, and improve boiler lifespan. In thermal systems, what goes up the stack reveals what’s being left behind in performance.
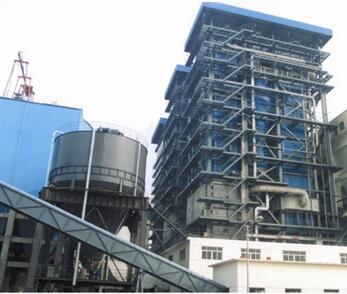
How Do Load Fluctuations and Turndown Ratio Affect Thermal and Combustion Stability?
In industrial boilers and combustion systems, demand for steam or heat is rarely constant. Load variations—caused by changing process demands, shifts, or seasonal conditions—require the burner to adjust its firing rate accordingly. If the system lacks the ability to modulate smoothly over a wide range, it becomes unstable during low-load periods. This is where turndown ratio plays a critical role: it defines the system’s flexibility to operate efficiently and stably at partial loads without frequent on-off cycling, flame instability, or thermal inefficiencies.
Load fluctuations and turndown ratio affect thermal and combustion stability by determining how effectively a boiler or burner can match its output to real-time demand. A low turndown ratio limits modulation and causes frequent cycling, leading to flame instability, incomplete combustion, and increased fuel use. A high turndown ratio allows the system to operate continuously at reduced loads, maintaining stable flame conditions, efficient fuel use, and consistent heat transfer. Properly managing load swings with appropriate burner design and controls is essential for maintaining stable and efficient operation.
The better your system can adapt to changing loads, the more stable, efficient, and reliable your combustion performance will be.
A high turndown ratio helps maintain combustion stability during load fluctuations.True
It allows the burner to operate continuously at low loads without shutting down, avoiding cycling-related inefficiencies.
🔄 What Is Turndown Ratio?
Turndown ratio is the ratio of a burner’s maximum firing rate to its minimum stable firing rate.
Burner Size (MMBtu/hr) | Minimum Stable Output (10:1) | Minimum Stable Output (3:1) |
---|---|---|
10 | 1 MMBtu/hr | 3.3 MMBtu/hr |
20 | 2 MMBtu/hr | 6.7 MMBtu/hr |
A 10:1 turndown burner can operate from 100% to 10% capacity continuously, while a 3:1 system must shut off if load drops below 33%.
📉 Thermal and Combustion Impacts of Load Instability
Condition | Impact Without Modulation | Impact With High Turndown |
---|---|---|
Low Load Demand | Burner cycles on/off frequently | Burner modulates to match load |
Rapid Load Swings | Flame instability, CO spikes | Smooth flame transition |
Extended Low Load Periods | Overheating and short cycling | Continuous, efficient firing |
Frequent Start/Stop | Mechanical wear, safety risks | Reduced maintenance, stable control |
Burner cycling not only affects combustion but also leads to thermal stress on components and uneven steam output.
Frequent cycling due to load swings improves burner reliability and reduces fuel use.False
Cycling increases wear and tear, raises purge losses, and reduces efficiency due to start-up fuel consumption and flame instability.
🔧 Why Stability Suffers During Load Fluctuations
Factor | Result of Inadequate Turndown | Stability Consequence |
---|---|---|
Inaccurate Air-Fuel Ratio | Over/under-oxygenated flame | CO formation, unburned fuel |
Incomplete Flame Development | At low loads, flame doesn’t stabilize | Flameout or burner lockout |
Delayed Re-ignition | Time gap between cycles | Output gaps, thermal shocks |
Over-firing After Restart | Burner ignites at full load | Steam pressure spikes |
Turndown flexibility prevents these problems by allowing the burner to gradually throttle down and up based on real-time demand.
📊 Performance Comparison by Turndown Ratio
Load (%) | Efficiency with 3:1 Turndown | Efficiency with 10:1 Turndown | Combustion Stability |
---|---|---|---|
100 | 88% | 88% | Stable |
75 | 82% | 86% | Moderate |
50 | 76% (cycling starts) | 84% | Stable |
25 | 68% (frequent cycling) | 81% | Stable |
10 | Not operable (off) | 78% | Stable |
High turndown ratios eliminate combustion drift and minimize efficiency loss at part loads.
🧠 Real-World Solution: Advanced Burner Controls
Feature | Function | Benefit |
---|---|---|
Parallel Positioning System | Separately controls fuel and air | Accurate air-fuel ratio across load range |
Oxygen Trim | Adjusts excess air in real time | Maintains combustion efficiency |
Modulating Controls (PID) | Adjusts firing rate smoothly | Prevents thermal cycling |
SCADA with Load Forecasting | Predicts load swings and adapts in advance | Maximizes uptime and efficiency |
Advanced burner modulation systems reduce the need for manual intervention during load changes.True
They adjust combustion parameters automatically to maintain optimal performance across varying loads.
🧪 Case Study: Steam Plant Turndown Upgrade
A 25 TPH gas-fired boiler at a university power plant suffered:
Frequent shutdowns during weekends
Flue gas O₂ spiking to 7–9%
Burner cycling 20+ times per day
Solution:
Burner retrofitted from 3:1 to 10:1 turndown
Added oxygen trim and PID modulation
Results:
Continuous firing down to 15% load
O₂ reduced to 3.2%
Efficiency gain: 6.8%
Annual fuel savings: ~$88,000
Burner life extended by ~2 years
📋 Best Practices for Load and Turndown Management
Practice | Goal |
---|---|
Burner Sizing for Lowest Load | Avoid oversizing that forces cycling |
High-Turndown Burner Selection | Enable flexibility across load ranges |
Real-Time Monitoring | Track and respond to O₂, CO, and load |
Regular Tuning | Ensure air-fuel curves are accurate |
Use of Multiple Boilers | Modular load sharing at high efficiency |
Modular systems with lead-lag control are especially effective in highly variable load environments.
Summary
Load fluctuations are inevitable—but combustion instability and efficiency losses don’t have to be. Turndown ratio defines how flexibly your boiler system can respond to real-time demand without cycling or overheating. A higher turndown ratio ensures smooth modulation, consistent flame control, and reduced fuel waste. Combined with advanced burner control technologies, operators can maintain combustion stability, minimize wear, and reduce operational risk across all load conditions. In modern thermal systems, adaptability isn’t just a convenience—it’s a core requirement for sustainable performance.
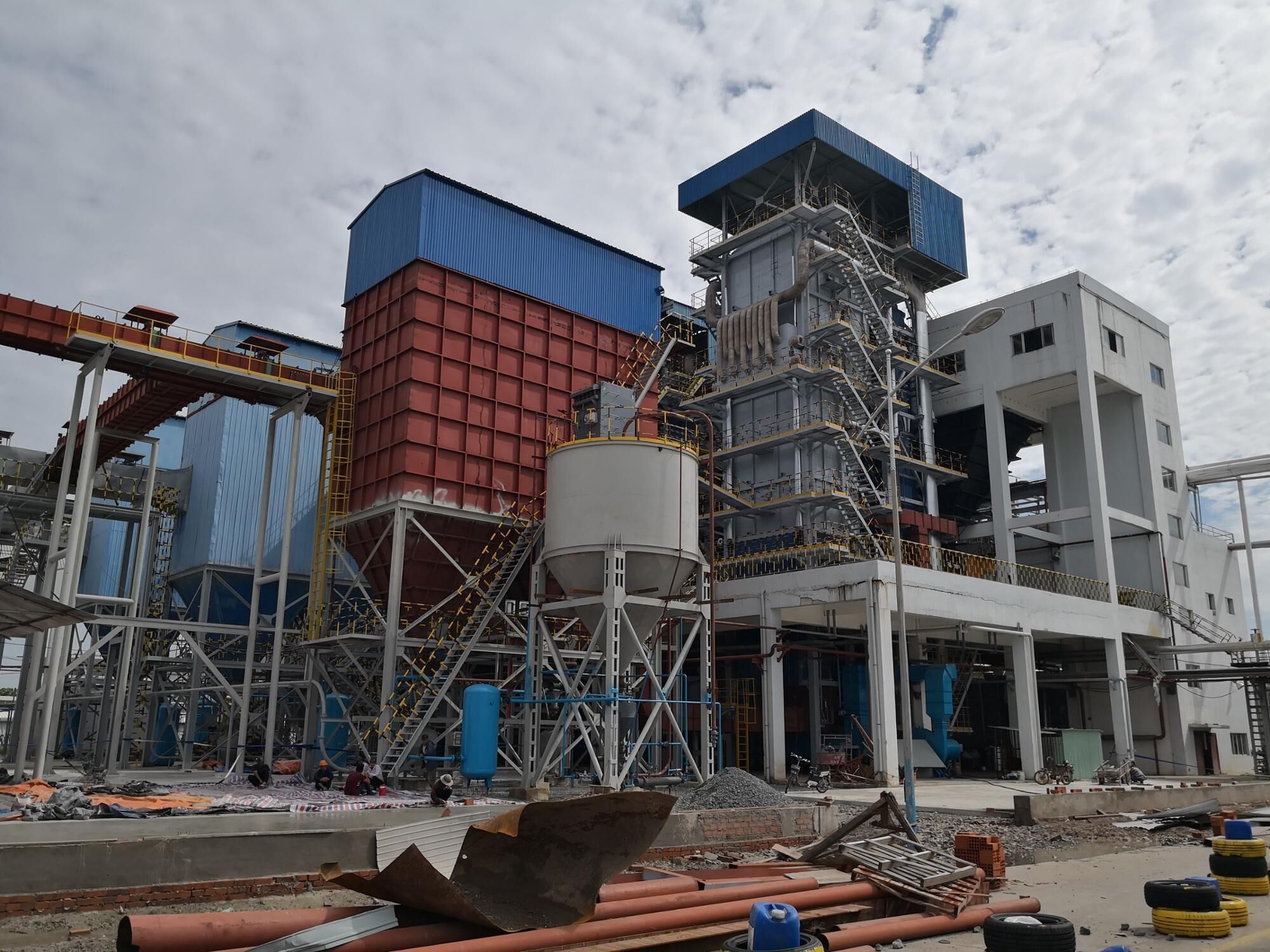
What Maintenance and Cleaning Practices Are Key to Sustaining Optimal Heat Transfer?
Heat transfer surfaces in boilers, heat exchangers, and furnaces are critical to efficient energy conversion. These surfaces are designed to maximize the flow of heat from combustion gases to water, steam, or process fluids. But over time, they become coated with soot, scale, slag, and other fouling materials that act as insulators, preventing effective heat exchange. If left unmaintained, these deposits cause energy loss, higher flue gas temperatures, increased fuel use, and even equipment damage. That’s why routine maintenance and proactive cleaning are essential for maintaining peak thermal performance.
Maintenance and cleaning practices are key to sustaining optimal heat transfer because they remove insulating deposits such as soot, scale, slag, and ash that reduce thermal conductivity. Regular cleaning of fire-side and water-side surfaces prevents efficiency losses, overheating, and unplanned downtime. Essential practices include soot blowing, chemical descaling, water treatment, thermal imaging inspections, and condition-based maintenance scheduling. These actions ensure that heat transfer surfaces remain clean, functional, and efficient—preserving fuel economy, output reliability, and equipment longevity.
In thermal systems, the more effectively you maintain heat transfer surfaces, the more energy you keep in the system—and out of the stack.
Soot and scale deposits on heat transfer surfaces significantly reduce boiler efficiency by increasing thermal resistance.True
Even thin layers of fouling insulate surfaces and force the system to burn more fuel to reach the desired output.
🔧 Common Fouling Materials and Their Impact
Fouling Type | Area Affected | Source | Efficiency Loss Potential (%) |
---|---|---|---|
Soot | Fire-side tubes | Incomplete combustion | 2–5% for 0.5 mm |
Scale | Water-side surfaces | Hard water (Ca, Mg, silica) | 10–15% for 1 mm |
Ash/Slag | Superheater, economizer | Biomass/coal ash fusion | 6–12% |
Corrosion Products | Both sides | Chemical reactions or oxygen ingress | Variable |
These fouling agents form thermal barriers, forcing boilers and heat exchangers to work harder—raising both fuel use and emissions.
📉 Impact of Fouling on Heat Transfer Performance
Deposit Thickness (mm) | Heat Transfer Reduction (%) | Flue Gas Temp Increase (°C) | Combustion Efficiency Loss (%) |
---|---|---|---|
0.3 (soot) | 2–4% | +25–30°C | 1.5–2% |
1.0 (scale) | 10–12% | +40–50°C | 3–6% |
2.0+ (mixed fouling) | >20% | +70–100°C | 7–10% |
Fouling can raise flue gas temperatures and cause significant stack heat loss.True
Insulating deposits prevent heat from being absorbed by the water/steam, pushing more heat out the stack.
🧰 Essential Maintenance and Cleaning Methods
Method | Target Surface | Description | Frequency |
---|---|---|---|
Soot Blowing | Fire-side tubes | Uses steam or compressed air to dislodge soot | Daily or per shift |
Chemical Descaling | Water-side tubes | Acid or alkaline treatment to dissolve minerals | Annually or as needed |
Mechanical Brushing | Tubes and superheaters | Manual cleaning of hardened deposits | During shutdown |
Ash Hopper Cleaning | Economizer/superheater | Removes accumulated ash manually or via rake | Weekly |
Online Cleaning Balls | Water-side systems | Circulate abrasive balls to clean tubes | Continuous (in some plants) |
In biomass and waste-fired systems, online and automatic cleaning is often required to manage high ash and slag loads without shutdowns.
🧪 Case Study: Chemical Plant Boiler Efficiency Restoration
A 25 TPH coal/biomass boiler at a chemical plant showed:
Flue gas temp: 275°C
Steam output down by 8.5%
O₂ levels high (6.5%)
Fuel consumption increased by 12%
Diagnostics:
0.6 mm soot on fire-side
1.3 mm scale on water-side
Economizer fouling observed
Actions Taken:
Soot blowing intensified to twice daily
Descaling with EDTA solution
Economizer manually brushed and flushed
Results:
Flue gas temp reduced to 185°C
Steam output restored to nameplate
Efficiency gain: 9.4%
Annual fuel savings: ~$72,000
Regular cleaning of heat transfer surfaces can lead to measurable fuel savings and output recovery.True
Cleaning removes insulating layers that block heat transfer, restoring thermal performance and reducing fuel consumption.
📋 Water-Side Maintenance Essentials
Task | Importance | Interval |
---|---|---|
Blowdown | Removes sediment and impurities | Daily |
Softener/RO Maintenance | Prevents hardness and silica ingress | Weekly |
Water Testing | Monitors pH, hardness, TDS, conductivity | Daily |
Descaling | Removes hardened scale deposits | Annually |
Deaerator Inspection | Prevents oxygen corrosion | Monthly |
Water chemistry is critical—even well-maintained boilers can scale quickly if water treatment lapses.
🧠 Monitoring Tools for Condition-Based Cleaning
Tool/Technology | What It Measures | Benefit |
---|---|---|
Flue Gas Temperature Sensors | Detect drop in heat absorption | Early indicator of fire-side fouling |
Differential Pressure Gauges | Track pressure drops across tubes | Fouling detection in economizers, preheaters |
Thermal Imaging Cameras | Surface temperature profiling | Identifies hot spots and insulation loss |
Ultrasonic Thickness Meters | Measures corrosion, internal scale | Tracks long-term wear and fouling buildup |
SCADA/Trend Monitoring | Detects gradual efficiency drift | Triggers cleaning before severe degradation |
Modern plants use these tools to implement predictive cleaning, based on actual system behavior rather than a calendar.
Summary
Optimal heat transfer is essential for high-efficiency combustion, and maintenance is the foundation of that performance. Soot, scale, slag, and ash build up over time, insulating key surfaces and lowering heat absorption. Through regular cleaning—both manual and automated—alongside water treatment and diagnostic monitoring, operators can maintain clean surfaces, efficient heat transfer, and low fuel use. In thermal systems, every layer you remove is energy you recover—and profit you protect. Sustained performance comes not from running harder, but from staying clean and running smarter.
🔍 Conclusion
Maximizing combustion and heat transfer efficiency in industrial power plant boilers requires a balanced approach to fuel quality, airflow management, thermal loading, and surface cleanliness. When these factors are well-optimized, the boiler operates more efficiently, consumes less fuel, and generates fewer emissions—all while maintaining output reliability. Regular inspections, predictive maintenance, and combustion tuning are essential for long-term success.
📞 Contact Us
💡 Want to improve the performance of your power plant boiler? Our specialists provide system audits, combustion optimization, heat transfer diagnostics, and efficiency improvement plans for high-capacity boiler systems.
🔹 Connect with us today to ensure your power boiler is running at peak efficiency and output! ⚡🔥📈
FAQ
How does fuel type influence combustion efficiency in power plant boilers?
Fuel properties such as calorific value, moisture, ash content, and volatility directly impact combustion. High-moisture or low-energy fuels require more air and heat to burn, reducing efficiency. Consistent, high-quality fuel improves flame stability and energy output.
What role does flame temperature play in heat transfer efficiency?
Higher flame temperatures promote more complete combustion and better radiation heat transfer to boiler walls and tubes. However, excessive temperatures can cause slagging, NOx formation, and material degradation. Balanced flame conditions are essential for both efficiency and equipment longevity.
Why is boiler design important for efficient heat transfer?
Efficient boilers have optimized surface area, tube arrangement, and flow paths to ensure maximum heat absorption from hot gases. Designs that reduce dead zones and promote turbulence in gas and water/steam flows enhance thermal transfer.
How do flue gas properties affect thermal performance?
High flue gas temperature indicates poor heat recovery. Efficient systems use economizers, air preheaters, or condensing units to extract residual heat. Monitoring oxygen levels, CO, and stack temperature also helps fine-tune combustion.
What operational factors affect boiler efficiency in power plants?
Air-to-fuel ratio tuning
Burner maintenance and calibration
Soot blowing and scale removal
Boiler load consistency
Real-time performance monitoring
Maintaining clean heat surfaces and precise combustion control is essential to maximize energy output and minimize fuel consumption.
References
Fuel Quality and Combustion in Boilers – https://www.energy.gov
Boiler Heat Transfer Optimization – https://www.sciencedirect.com
Power Plant Boiler Design and Efficiency – https://www.researchgate.net
Combustion Control in Industrial Boilers – https://www.epa.gov
Thermal Performance of Steam Generators – https://www.iea.org
Economizer and Heat Recovery Systems – https://www.bioenergyconsult.com
Burner Management for Large Boilers – https://www.mdpi.com
Boiler Maintenance and Performance Monitoring – https://www.automation.com
Steam System Optimization in Power Plants – https://www.energysavingtrust.org.uk
ASME Guidelines for Boiler Efficiency – https://www.asme.org
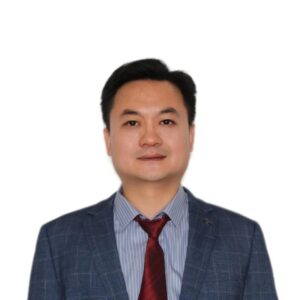